Considerations for Protecting Cell Viability During Cryopreservation
Cryopreservation extends the viability of cells by cooling them to extremely low temperatures and thus keeping them in a safe, biochemically inactive state. This process gives much-needed flexibility to researchers and those developing cell-based therapies. The latter involves collecting living cells from donors, transporting them to manufacturing sites for processing or screening, and then delivering the product to the patient. Cryopreservation enables “you to separate your upstream and downstream operations in time and location,” says Dr. Katie Pollock, Associate Director and Head of Formulation and Cryobiology at Bristol Myers Squibb.
Bringing a cell suspension to and from a cryogenic state must be done carefully, as cells can be easily damaged by the formation of ice crystals forming in subzero temperatures. The cryopreservation process can be broken into five steps: formulation, freezing, storage, thawing, and post-thaw handling (Figure 1). “It’s important to understand that the process is the product. What you do along this path influences the quality at the end,” says Dr. Allison Hubel, Professor of Mechanical Engineering at the University of Minnesota and President-elect of the Society for Cryobiology.
In this article, we’ll discuss why the science of cryopreservation is so complex and describe how to minimize cell injury throughout the process.
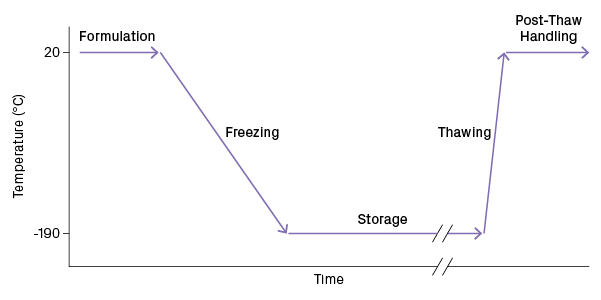
Figure 1. Cryopreservation process. Cells are prepared, frozen, stored at cryogenic temperatures, thawed, and then used for downstream processes. Adapted from Dr. Pollock’s presentation at the Cryo Symposium.
Mechanisms of Cell Damage During Freezing
A cell suspension is a complex mixture, and its freezing occurs over a range of temperatures. Let’s consider what happens to cells immersed in a cryopreservation solution as they are gradually cooled from ambient to cryogenic temperatures (Figure 2). When the temperature drops several degrees below 0°C, water molecules begin to crystalize into ice. As cooling continues, the ice crystals grow and the fraction of liquid water decreases. The presence of solutes prevents the entirety of water from freezing into ice. Cells become trapped in the narrowing channels between crystals and lose water due to the rising osmolarity of the surrounding solution.
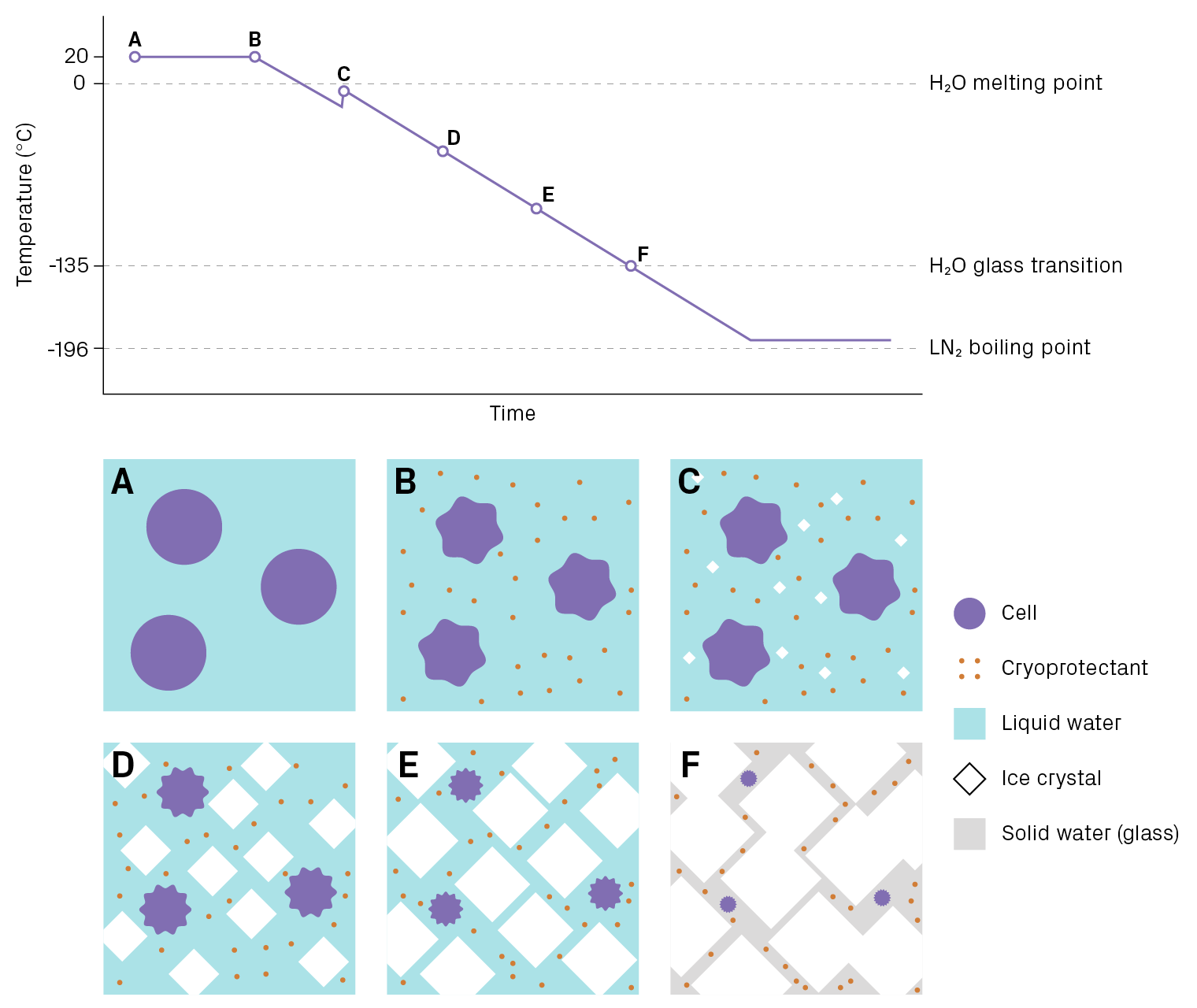
Figure 2. Controlled-rate cooling of a cell suspension. (A) Cells are collected. (B) A cryopreservation medium is added, and cells shrink due to the hypertonic solution. (C) Ice nucleates. Since crystallization is exothermic, the temperature jumps briefly. (D-E) The ice crystals grow, and solute concentration increases in the shrinking liquid portion. As a result, cells continue to lose water and volume. (F) The remaining liquid water solidifies into a glass. Further cooling does not change the structure of the mixture. Adapted from Dr. Pollock’s presentation at the Cryo Symposium.
Once the temperature reaches about -135°C, the remaining liquid water (inside and outside the cell) hardens into a non-crystalline or amorphous solid, more commonly known as a glass. In this state, water is completely immobilized and cannot participate in chemical reactions, which prevents biological molecules from degrading. If kept below -135°C, cells can remain unchanged indefinitely. (The term “cryogenic” usually refers to temperatures below -150°C.) However, cells face at least three dangers as they traverse this large temperature range:
- Intracellular ice. The formation of large ice crystals inside the cell is usually lethal. Intracellular ice is thought to damage cellular structures such as organelles and the cytoskeleton1,2.
- Osmotic stress, aka “solution effects” injury. As liquid water gets locked into ice, the solute concentration of the media increases, causing water to exit the cell. The degree of dehydration is dramatic: cells lose about 90% of their water3. The high osmolarity can denature proteins and disrupt membranes.
- Mechanical forces from extracellular ice. As the ice crystals grow, cells are squeezed into the gaps between crystals. This mechanical stress can damage cells and impact viability.
A successful cryopreservation strategy uses a combination of tools to mitigate these risks, including cryoprotectants, controlled cooling and thawing rates, and reliable storage units. We’ll discuss the role of each below. Keep in mind that cryopreservation protocols must be tailored to the cell type, which has a particular composition and sensitivity to stress.
Automated systems designed to manage cell temperature effectively and minimize transient warming support a successful cryopreservation strategy. One example is the new Cryo Store Pico™. Read more >
Formulation: Introducing the Cryopreservation Solution
Cyroprotectants, or cryoprotective agents, are solutes that can protect cells from damage during cryopreservation. They are added to cell suspensions prior to freezing. The first cryoprotective agent, glycerol, was discovered in 1949 by chance4. Since then, dozens of compounds have been found to have cryoprotective properties, including dimethyl sulfoxide (DMSO) — a popular choice for mammalian cells5.
How do they work? Roughly speaking, cryoprotectants reduce ice formation during freezing, keeping a larger portion of the extracellular solution unfrozen at any given temperature. As such, cells experience less dehydration and osmotic stress. The exact mechanism of action is not fully understood and varies by substance.
The initial step of cryopreservation is formulation, which involves adding a cryopreservation medium to harvested cells. There are two risks to cell health at this stage.
- Cell volume changes (osmotic shock). The cryopreservation medium is hypertonic to the cells and will cause them to lose water.
- Cryoprotectant toxicity. Although cryoprotectants are chosen for their low toxicity to cells, they can still interfere with biological processes during long exposures, especially at warmer temperatures. Changes in cytoskeleton organization, metabolism, and membrane composition have been observed in affected cells6.
It’s recommended to pre-chill cryopreservation medium and add it to cells gradually. Under these conditions, cell shrink more slowly and cryoprotectant toxicity is reduced. “By stretching those operations out, you can be kinder to your cells and cause them less cell death or damage or stress during the formulation process,” explains Dr. Pollock. Once the cryopreservation solution is fully introduced and cells are equilibrated, freezing should begin as soon as possible. Delays can worsen the toxic effects of the cryoprotective agent.
Freezing: Not Too Fast, Not Too Slow
The rate of cooling greatly influences when and where ice forms in the mixture. Rapid cooling doesn’t allow enough time for water to leave the cell, causing intracellular ice to form. Slow cooling overly dehydrates cells, making them vulnerable to osmotic stress. An optimal rate will balance these risks to minimize cell damage (Figure 3). For many mammalian cell suspensions, a cooling rate of 1°C per minute is recommended, which can be carried out with a cell freezing container.
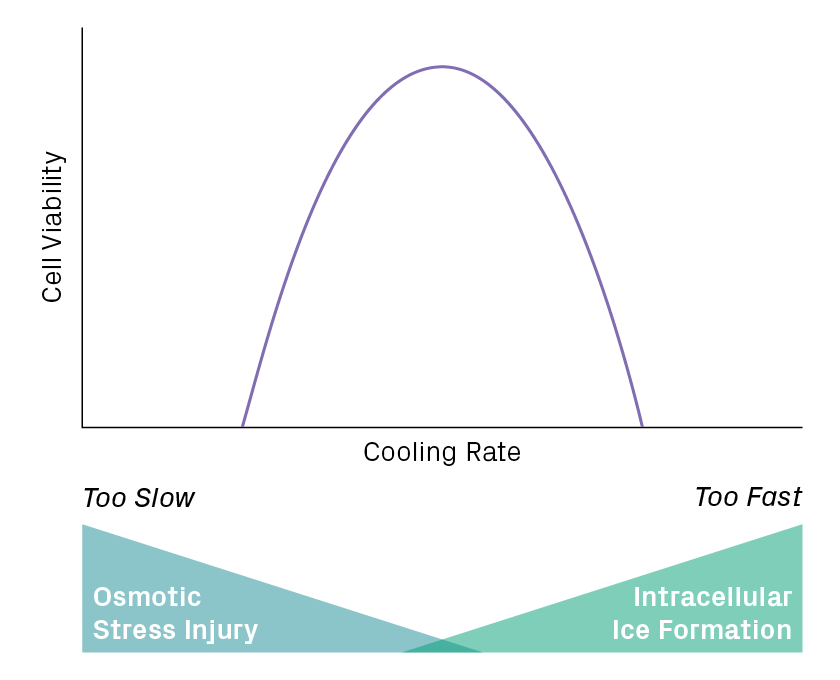
Figure 3. Effect of cooling rate on cell viability in an idealized example. An optimal rate of cooling minimizes the total damage from osmotic stress and intracellular ice formation. Adapted from Dr. Pollock’s presentation at the Cryo Symposium.
Storage: Watch Out for Transient Warming
Keeping materials at cryogenic temperatures (-150°C and below) is usually accomplished with liquid nitrogen (LN2), which has a boiling point of -196°C. Materials can be immersed in the liquid phase or kept in the vapor, the latter being only slightly warmer (about -190°C) yet less prone to contamination and much safer to work with. Long-term storage or shipping is possible at this stage, provided the materials maintain a temperature below the glass transition point of water (approximately -135°C). Warming above this threshold triggers microscopic melting and recrystallization, endangering cell integrity because larger ice crystals tend to grow at the expense of smaller ones7.
Even brief temperature excursions can be pernicious, especially if they occur repeatedly. These events are commonplace during routine operations such as retrieving materials from a freezer. When someone opens a freezer and pulls out a rack to locate a sample, the entire contents of the rack are exposed to ambient temperatures. Rapid warming ensues. If the task takes longer than a minute or two, materials may warm above -135°C. For cell and gene therapies, the stakes are high. “It's a real thing that transient warming negatively affects product quality, viability, and potency and is a potential risk to patients who would see this product,” says Dr. Jason Acker, a Professor in the Department of Laboratory Medicine and Pathology at the University of Alberta.
The risk of cell injury from transient warming can be reduced by training staff to work quickly and efficiently when handling cryopreserved materials. But the most effective strategy is to eliminate human intervention where possible. Automated LN2 freezers use robotics to locate and retrieve materials, which makes the process highly reproducible and less susceptible to transient warming events.
(Intentional) Thawing of Cryopreserved Cells
When cells are ready for downstream use, they must be thawed. As discussed above, warming past the glass transition temperature of water sets the stage for ice recrystallization, namely the growth of larger and more dangerous crystals. Therefore, cells should be exposed to these conditions as briefly as possible. Warming rates of 60°C to 80°C per minute are usually recommended and can be achieved with controlled thawing devices.
When working with cell therapy products, it’s essential to consider how thawing is performed at three locations: manufacturing, quality control, and clinical sites. “You want to ensure comparability between your QC samples and your…final drug product,” says Meiling May, Principal Scientist at Novartis. “There’s often a discrepancy because when you think about the volumes used to infuse your drug product, you often don’t want to waste precious cell material [by] sending that whole volume as well to QC.”
Post-Thaw Assessment
Measuring cell health after thawing is vital to ensure the usability of cells for downstream applications and to assess the efficacy of the preservation process. The choice of assays and how you perform them matters greatly. “It’s easy to do post-assessment poorly. There was a program in Kansas City that ‘improved’ their preservation process. [It resulted] in the death of a fourth of the patients…because they used an inappropriate post-thaw assessment methodology,” says Dr. Hubel. Viability assays that rely on dye exclusion from cells, such as trypan blue, are commonly used. However, they only test for membrane integrity and fail to detect other relevant damage, such as metabolic changes or apoptosis. A combination of assays is the best approach to obtain a comprehensive and accurate assessment of cell health7.
Conclusion
Cryopreservation requires a rigorous workflow to protect cells reproducibility. New findings from cryobiology are enhancing our understanding of cell preservation and improving the tools used to carry it out. To learn the latest developments in the field, view the recorded talks from the inaugural Cryo Symposium for Cell & Gene Therapies.
References
1. Acker, J.P. & McGann, L.E. Membrane damage occurs during the formation of intracellular ice. Cryo-Letters. vol. 22 241–254 (2001).
2. Müllers, Y. et al. Quantitative analysis of F-actin alterations in adherent human mesenchymal stem cells: Influence of slow-freezing and vitrification-based cryopreservation. PLOS ONE vol. 14 e0211382 (2019).
3. Mazur, P. Freezing of living cells: mechanisms and implications. American Journal of Physiology-Cell Physiology vol. 247 C125–C142 (1984).
4. Polge, C., Smith, A. U. & Parkes, A. S. Revival of Spermatozoa after Vitrification and Dehydration at Low Temperatures. Nature vol. 164 666–666 (1949).
5. Elliott, G. D., Wang, S. & Fuller, B. J. Cryoprotectants: A review of the actions and applications of cryoprotective solutes that modulate cell recovery from ultra-low temperatures. Cryobiology vol. 76 74–91 (2017).
6. Meneghel, J., Kilbride, P. & Morris, G. J. Cryopreservation as a Key Element in the Successful Delivery of Cell-Based Therapies—A Review. Frontiers in Medicine vol. 7 (2020).
7. Baust, J. M. Molecular Mechanisms of Cellular Demise Associated with Cryopreservation Failure. Cell Preservation Technology vol. 1 17–31 (2002).